First axion results from the XENON100 experiment
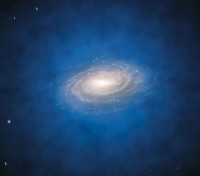
Paolo Beltrame of the School's Nuclear Physics group explains the background to his co-authored paper which appears in this month's Physical Review D.
Which is better: a dark matter WIMP or the Imp from GoT (Game of Thrones)? I don’t know, but I advise you not to forget the axions from GUT (Grand Unification Theories). Axions, if they exist, could solve several problems in understanding our universe and in the description of the forces that govern the subatomic world.
Axions were first postulated by Roberto Peccei and Helen Quinn in 1977 to explain the discrepancy between theory and observation in Quantum Chromodynamics in relation to the Charge-Parity Violation. They could be an excellent dark matter candidate and could also solve the CPV problem. But what does this mean?
In the Standard Model of particle physics, the fundamental force that regulates the interaction among quarks is called the Strong Force. Quarks are thought to be the fundamental constituent of hadrons, among which we have the nucleons, ie the protons and neutrons which make up atoms. Each type of quark is named after a colour. This doesn’t mean that quarks are literally red, green or blue: the colours are just a naming convention to distinguish the different kinds of quarks. Because of these colours, the quantum theory formalism that describes the quarks gets the name of chromo: Quantum Chromo Dynamics or QCD.
The Weak Force
Now, in the Standard Model we have another force, called the Weak Force. This Weak Force is responsible for the decay of the nuclei; and whenever a neutrino is involved. Why do we care about Weak Interaction if the axions deal with the Strong one? This is because of the CP symmetry violation.
In 1964 it was found that the Weak Interaction violates the CP symmetry. The fundamental particles may come with a charge (C), like the electron, and with a parity (P), which can be seen as a spatial symmetry. Like the human face which is symmetrical (although not perfectly so) between left and right. Before 1964 it was expected that by changing the charge of a particle (performing a so-called charge conjugation) you would get something different from what you had at the beginning. So a positron is not an electron, but is instead its charged-conjugated partner. The same thing was expected to happen with the parity conjugation: imagine putting a particle in front of a mirror, the mirrored particle won’t be the same as the original one.
However, it was believed that if you combine these two transformations (if you make a CP conjugation) you obtain the same situation as the one present at the beginning of the process. Well, in 1964, it was proven that this is not the case for the Weak Interactions, that is to say Weak Interactions violate the CP symmetry. Nowadays we understand this process better and we can precisely describe this violation within the Standard Model of particle physics. This CP symmetry violation, although perfectly fine with the Standard Model, has not been observed in the Strong Interaction. Imagine that you see a leaf that is about to fall from a branch, but never falls. The fall is predicted by gravity but it doesn’t happen, therefore we must be missing something like the leaf being stuck to the branch. So, what is it happening to the Strong Interactions? Why haven’t we yet observed the CP violation in the Strong sector of the Standard Model?
We don’t know… yet.
To solve this problem Peccei and Quinn introduced this new particle, the axion, that takes away the CP violation in the Strong Interaction processes, restoring the symmetry. It is like preventing the leaf from falling, and making the violation invisible.
Why is this important for us?
Simple. Now that the Higgs boson has been discovered and we have a clearer idea of how particles acquire mass, we are still unable to explain why we are living in a matter-dominated universe rather than an antimatter-dominated one. The definition of what is matter and what is antimatter is a purely human convention: the two options, matter or antimatter universes, would be completely indistinguishable in terms of the laws of nature. The only difference you might experience is that instead of electrons flowing when you switch on the light, you would do the same using positrons instead.
So why has Nature chosen matter (electron) instead of antimatter (positron)?
We think the solution lies in understanding the CP violation, and the axion is one of the keystones in the building of this cathedral. There are several experimental groups searching for these particles, and many theoretical physicists are working on various axion models (oscillating between predictions and readjustment, as experimental results are published).
Concerning the experimental searches, it was recently realised that the dark matter detectors (like CDMS, EDELWEISS), especially xenon-based instruments, are particularly suited to this challenge. So the dark matter community has involved itself in this venture.
Supported by several theoretical models (also arising from Grand Unification Theories), we expect the axions to interact with the normal baryonic matter by coupling with photons, nucleons or electrons. By normal baryonic matter we mean the building blocks that constitute the Universe with which we naturally interact. Everything you see, everything you touch, is normal baryonic matter. Also our detector is made of only baryonic matter, because this is the only thing that we have learnt to master. Nevertheless, our standard matter could allow us to detect the axions by using the so-called axio-electric effect, testing the probability that an axion will interact with an electron of our detector target (see Fig 1 below), kicking it out.
The axio-electric effect is very similar to the photo-electric effect (whose discovery won Albert Einstein the Nobel Prize for Physics in 1921), with one crucial difference: in our case instead of a photon we consider an axion, which hits the electron and ionises the xenon target. The axio-electric effect was first introduced and formalised by A. Derevianko and others in the late 1990s. This was understood and initiated by me, while I was a member of the XENON collaboration.
What happens when an axion hits the xenon target?
It generates a small spark, immediately detected by the photomultiplier tubes that continuously monitor the situation inside the detector.
The XENON100 detector has been particularly good at discovering the axions through this effect. The secret lies in the cleanliness of the detector. Dark matter detectors, particularly the ones based on Xe, are among the cleanest places in the Universe. In what way? Everything surrounding us is radioactive, emits radiation which continuously hits us: even when you wash your hands you receive some radiation, particularly if the washbasin is made of ceramic because of the cobalt it contains. This radiation is completely harmless for your body so we never worry about it. But in contrast, if you put the same amount of ceramic inside a dark matter detector, the whole experiment would be spoiled! Hence, every single component has been carefully selected and the detector is operated in such a way that everything that generates a spark in its interior can be considered as a good signal, and not some spurious radiation.
To give you an idea of the cleanliness of the XENON100 detector: imagine you could sit inside the inner part of it (wearing the proper clothes, since the temperature is about -100 degrees). That place is so radiation-clean that you will have to wait for about a day between one low-energy event (visible as a tiny spark) and another. This means that if we see some light there is a good chance that it is coming from something interesting — such as axions.
We ran an experiment in the XENON100 detector for more than a year, then skimmed the data that we had collected during that time. At the end of the skimming procedure we have found no evidences of axions, as shown in Fig 2 below.
We have performed two different searches. One targets the axions which are emitted by the Sun (also called solar axions), which then reach the Earth at high velocity, ie energy, and interact within the detector. The results are shown on the left plot of Fig 2. The other search looks for axions that are slowly moving within our galaxy. Our planet, and the XENON detector, passes through this cloud of axions, possibly causing an interaction with the target. The outcome of this second approach is shown on the right plot of Fig 2.
How to read these graphs?
Consider the plot on the right, the one referring to the galactic axions. The y-axis shows the coupling of the axion with the electron, ie a way to describe the probability that they interact with the electrons. The x-axis shows the hypothetical mass of the axion. Since we don’t know either the coupling nor the mass, we have to plot them in such a graph to check where they like to live (for a given mass the corresponding coupling and vice-versa).
In these so-called exclusion plots, we show different experiments (whose names you can find on the plot) which have excluded certain phase space: each point [coupling, mass] above the line for a particular experiment has been rejected, and if the axion exists, it can be only in the region below these lines. For example, it is highly impossible that an axion in the galaxy can have a mass of 2 keV and a coupling to the electrons 1E-11 (ie one in eight hundredth of millionth), since these characteristics have been excluded by CoGeNT, CDMS, EDELWEISS and more recently by XENON100. An axion with a mass of 2 keV and a coupling of 1E-13 is still possible: we haven’t been able to search for that yet.
You can think of it like fishing: we try to sink our fishing lines deeper and deeper into different parts of the lake. You can immediately see that the XENON100 has reached the deepest level of this search. Similarly you can read the plot on the left, on which you can see different experiments and the XENON100 results. Other non-dashed lines represent constraints based on astronomical observations. In particular, the DFSZ and KSVZ refer to the theoretical models that allow the axions to solve the strong CP problem that I mentioned at the beginning.
For further and more technical information you are invited to read the original paper: 'First axion results from the XENON100 experiment' (Phys. Rev. D90, 062009 (2014), doi: 10.1103/PhysRevD.90.062009).
The effort to obtain the results from the XENON detector, which are presently world-leading, was led by myself in collaboration with the Weizmann Institute of Science. I am now a researcher at the School of Physics & Astronomy within the Dark Matter Direct Search group, along with Alex Murphy, James Dobson, Thomas Davidson and Maria Francesca Marzioni.
The University of Edinburgh has key roles within the LUX and LZ collaborations, which are the leading forces in dark matter research. The greater capability of the LUX detector means we can expect the quality of its results to exceed those of the XENON100 by a factor of five. And... perhaps discover the elusive axions!
It took 40 years to find the Higgs boson. The search for the axion has just begun and Edinburgh is leading the hunt for these fundamental, elusive particles.